The Origin of the Mitochondrion
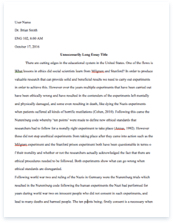
- Pages: 18
- Word count: 4351
- Category:
A limited time offer! Get a custom sample essay written according to your requirements urgent 3h delivery guaranteed
Order NowCells of almost all eukaryotic organisms contain mitochondria. These organelles are vital to the process of aerobic respiration, the conversion of organic molecules to energy in the form of ATP. l. In some cases, there is a single, large mitochondrion, but more often, there are hundreds or thousands in a cell. The number is generally correlated with the metabolic activity of the cell. These and other membrane-bound organelles are not found in prokaryotic cells (those that lack a nucleus), though these more primitive organisms have respiratory membranes that are quite similar to those in mitochondria.
This suggests that the mechanisms are very similar, leading to interest in tracing the evolutionary origins of the modern mitochondrion. In order to accomplish this, this paper focuses on the explanation of mitochondrial origins using molecular, genetic, and evolutionary approaches. There are very few papers that combine these approaches, often making it difficult to understand and compare the theories of mitochondrial origin.
The following will present a variety of opinions on how endosymbiosis attempts to bridge the gap between the organization of prokaryotic and eukaryotic cells and will highlight the evolutionary significance of anaerobic eukaryotes that do not have mitochondria. Endosymbiotic Theory The original theory behind the origin of the organelles in a eukaryotic cell involves the infolding and specialization of the cell’s membrane, a process known as direct filiation (Margulis 1981). This theory is reasonable from an evolutionary standpoint since it is a process involving selection-driven modifications, the basis of evolutionary theories.
It does not, however, explain the similarities between mitochondria and free-living bacteria, nor does it account for the large gaps between aerobic and anaerobic organisms appearing in the fossil record (Schwartz and Dayhoff 1978). A more appropriate explanation of the evolution of eukaryotic cells is the endosymbiotic theory. First proposed by C. Mereschkowsky in 1905, the endosymbiotic theory was thought to account for the similarities between plastids and cyanobacteria. He suggested that chloroplasts were once free-living photosynthetic bacteria taken into a larger host cell (Martin et al. 001).
In 1927, American biologist I. E. Wallin argued that in appearance, behaviour, and response to stains, mitochondria were so similar to bacteria that they must have once been a similar organism, somehow incorporated into the cytoplasm of another cell that became the modern eukaryote (Margulis 1981). Because of an early lack of solid genetic and molecular evidence, the endosymbiotic theory was given little attention until the 1970s, when American molecular biologist Lynn Margulis explored the subject in great detail and developed the current hypothesis (Margulis 1978 and Margulis 1981).
Endosymbiosis” refers to the relationship in which one cell lives inside a larger cell while the two exchange resources such as food, shelter or energy (Cooper 1997). According to the endosymbiotic theory for the origin of mitochondria, the organism that became the mitochondrion, the endosymbiont, had the ability to carry out oxidative phosphorylation to obtain energy. This cell was incorporated into the cytoplasm of a larger anaerobic cell (the host), and was not digested (Becker et al. 2003).
Through various mechanisms the two cells managed to survive together, and evolved to be fully dependent on one another-a mitochondrion cannot survive outside the cell, and a cell with mitochondria cannot survive if they are removed (Margulis 1978). A question that should be raised at this point is whether or not such an obligate symbiosis between two distinct organisms can be proven. American microbiologist Kwang Jeon and his colleagues (1997) have developed adequate proof that a symbiosis between the primitive mitochondrion and its host could have been possible.
Working with strains of Amoeba proteus, Jeon introduced a harmful strain of X-bacteria to the cells in the study. Many of the infected amoebas were killed by the infection, but some managed to survive with populations of bacteria still living inside them. Over time, the surviving infected cells became dependant on their endosymbionts, the once poisonous bacteria. If Kwang removed the endosymbionts surgically, the amoebas would not survive. He discovered that this was because the infected amoebas would stop producing an enzyme that is vital for survival.
When the symbiosis occurred, the bacteria began to provide the amoebas with the missing enzyme, forcing them to be fully reliant on their endosymbionts for survival, much to the advantage of the bacteria. Though it does not necessarily comment on mitochondrial origins, this experiment suggests that the endosymbiotic theory is indeed physically possible. The next step to discovering the origin of the mitochondrion is determining the identities of the free-living symbiont and host, as well as the mechanisms that instigated and maintained the association.
The Endosymbiont The original free-living form of the mitochondrion is thought to have been a rod-shaped, Gram-negative aerobic proteobacterium (Becker et al. 2003 and Gray 1996). As the endosymbiosis is thought to have occurred shortly after oxygen became prevalent in the atmosphere, organisms that could use the newly abundant oxygen in respiration would have a great advantage over those who could not. Oxidative phosphorylation, the use of oxygen in respiration, generates a significantly larger amount of ATP than fermentation alone.
Thus, the purple bacterium would have previously developed the Krebs-cycle enzymes and the cytochrome system necessary for total oxidation of organic compounds, making its energy-harvesting system much more efficient than other organisms of the time (Margulis 1981). The similarities between mitochondria and bacteria provide startling evidence for the idea that mitochondria were once free-living. Most noticeably, bacteria and mitochondria are approximately the same size (1-10 microns); they have similar shape, and respond in the same way to stains (Sengbusch 2002).
Mitochondria are observed dividing by the bacteria-like binary fission, not by the mitosis of eukaryotes, and divide independently of the host cell (Gooch 2002). Another similarity is that both bacteria and mitochondria have circular DNA that lacks associated histone proteins, attached to their inner membranes (Fairbanks and Anderson 1999). Each have 70S type ribosomes, as opposed to the 80S ribosomes in eukaryotes, and as a result, antibiotics such as chloramphenicol that kill many bacteria actually affect the protein production of mitochondrial ribosomes (Sengbusch 2002).
The inner membrane of the mitochondrion contains certain lipids that are only found elsewhere in bacterial membranes. The outer membrane, assumed to have been supplied by the host cell, does not differ from that of other eukaryotic cell membranes (Margulis 1981). Thus, it is not difficult to accept the concept of a once free-living bacterial “protomitochondrion. ” Perhaps a more daunting task is determining which aerobic bacterium was involved in the original symbiosis.
According to Margulis (1981), the most probable ancestor of mitochondria are aerobic bacteria capable of penetrating a host and replicating inside, such as Gram-negative proteobacteria Paracoccus denitrificans. The basis of this argument is the great similarity in the highly detailed respiratory systems of Paracoccus and the mitochondria of animals and yeast. The quinones and cytochromes of the electron transport chains, as well as their three-dimensional spatial configuration are so similar that Margulis suggests that P. enitrificans is more similar to mitochondria than any other bacterium (Margulis 1981).
One large difference between the two groups is that P. denitrificans lacks the ATP transport chain of mitochondria. Margulis claims that releasing ATP into its surroundings would not initially be beneficial to the bacterium, and that the ATP transport system must have evolved with the symbiosis in order to feed the host cell. More recent sequencing of 16S mitochondrial rRNA has allowed researchers to determine that the bacterial group most closely related to the present mitochondrion is the ? division of the proteobacteria (Martin 2000-2).
The most mitochondria-like eubacterial genome known is that of Rickettsia prowazekii, a Gram-negative obligate intracellular bacterium that is a causative agent of typhus (Lang et al. 1999). The relationship between Margulis’s proposed Paracoccus denitrificans and the more recently accepted Rickettsia prowazekii has not been explored by other researchers, most likely because the organisms have been chosen based on two different standards: molecular versus genetic.
As a member of the proteobacteria, Rickettsia may indeed share the same respiratory characteristics as Paracoccus. Also, the entire Paracoccus genome has not yet been sequenced and compared to the modern mitochondrion, lending some ambiguity to the comparison. Therefore, this paper proposes that both theories could point to a very similar organism that took part in the symbiosis to form the protomitochondrion. The Host In order for the protomitochondrion to take up residence in the cytoplasm, there must have existed a host that was able to acquire an endosymbiont and provide it with suitable living conditions.
The host for the origin of the mitochondrion is traditionally envisioned as a wall-less microbe that could ferment glucose to two- and three-carbon end products without the use of oxygen. In order to survive, the organism must have been able to cope with the growing level of atmospheric oxygen of the time (Margulis 1981). One problem with suggesting a prokaryotic host, however, is that no currently living prokaryote is known to have another organism living inside of it.
Margulis has therefore suggested that before symbiosis, a prokaryote folded some of its plasma membrane inward to encircle its DNA, making it a “protoeukaryote” (Margulis 1978). This primitive eukaryote, which would have a nucleus but lack other organelles, would have been the host for the symbiosis. This organism should have descendants alive today that primitively lack organelles such as mitochondria. Unfortunately, no such organisms have been found because contemporary protists lacking organelles may have secondarily lost their mitochondria when not needed in an anaerobic environment.
This was discovered when gene sequencing identified some remnants of mitochondrial genes in organisms such as Pelomyxa palustris, once thought never to have contained mitochondria (Martin 2000). Since these organisms were once thought to be the protoeukaryote’s ancestors, it is now quite difficult to identify a possible endosymbiotic host. Margulis predicted this very result in 1978, when she suggested that it was uncertain whether the organisms without mitochondria had never contained them, or that they lost these organelles if not needed.
Nevertheless, in the 1970s, researchers proposed the most commonly accepted candidate for a living descendant of the protoeukaryotes. This organism, Thermoplasma acidophila, is a heat-and acid-tolerant archaebacteria living in very extreme conditions, perhaps similar to those of the early earth (Margulis 1981). Lack of cell walls, an oxygen-independent fermentation pathway, and the possibility that relatives of this group have the ability to ingest particles such as other cells support the idea that this organism could be a descendent of the host (Margulis 1981).
Since there are no eukaryotes found to date that are missing all traces of mitochondrial genes, the identity of the host has yet to be confirmed. At this point, the largest problem with the endosymbiotic theory is the questionable identity of the symbiotic host; so far Thermoplasma has been the only organism suggested. The Mechanism of Endosymbiosis The measure in determining how a free-living bacterium became the mitochondrion is to examine how the symbiosis took place.
The most popular theory to explain this phenomenon is that the protoeukaryotic host must have ingested the aerobic protomitochondrion through a feeding process known as phagocytosis, or “cell eating” (Gooch 2002). If this were the case, then the matter of why the protoeukaryote did not digest its prey must be addressed. It may be possible that the protoeukaryote was in the habit of swallowing up and not digesting particulate organisms, since all living prokaryotes take up dissolved organic molecules directly from the environment, and need not engulf particles (Margulis 1978).
This hypothetical organism would not have existed unless it retained the ability to absorb molecules from the environment as well as phagocytosis, a phenomenon not observed in modern cells. Most likely, the protoeukaryote suffered a mutation that disabled its ability to digest engulfed particles, and the mitochondrion remained inside. The symbiosis itself may then have triggered the protoeukaryote to develop different ways to digest dissolved as well as engulfed particles, in order to feed itself and its symbiont.
Margulis (1981) claims that since all prokaryotes lack the ability to carry out phagocytosis, the protomitochondrion would not have been engulfed at all. Instead, she suggests that the initial association may have begun with the protomitochondrion preying on the host. The bacterium would have invaded the host and reproduced itself inside, becoming the endosymbiont that supplied energy to the host if it did not kill it. This concept supports Margulis’ idea that the obligate parasite Paracoccus denitrificans was the endosymbiont.
Finally, in order to understand how the symbiosis may have occurred, it is vital to decide whether the association took place only once (a monophyletic origin) or if an endosymbiosis between the two cells occurred several times, each with similar results (polyphyletic origin) (Margulis 1978). The fact that the mitochondria of plants, animals and fungi are very similar in appearance and function shows that the protomitochondria were acquired symbiotically before differentiating into the various kingdoms, and before photosynthetic plastids had been acquired.
Hence, the common ancestors of all eukaryotic cells were heterotrophic, mitochondria-containing protoeukaryotes, demonstrating the monophyly of mitochondria (Schwartz and Dayhoff 1975). Also, recent rRNA and protein studies have shown vast genetic and molecular similarities between mitochondria of several species, all of which have mitochondrial DNA that is a subset of that of Reclimonas Americana, an ancient organism with the greatest number of mitochondrial genes (Blackstone et al. 1995).
It would not be reasonable to suggest that these similarities could exist if several separate endosymbioses occurred. There should be more genetic variation if this were the case, as the mitochondria in different organisms evolved according to their own environmental pressures. Therefore, it is apparent that the endosymbiosis that resulted in eukaryotic cells with mitochondria must have happened only once, and all other eukaryotic cells have descended from the original partnership. Maintaining the Symbiosis
Once the protomitochondrion was incorporated into the host’s cytoplasm, there must have been some mechanism to allow the continuation of the endosymbiosis. In other words, there must have been some selection that would have favoured the propagation of an anaerobic protoeukaryote that had an aerobic symbiont living inside of it. The first possible scenario to explain such selection is that the endosymbiont used atmospheric oxygen in order to produce a large amount of ATP for both itself and the host, in exchange for food and shelter, a rather benign interaction (Blackstone 1995).
Another scenario focuses on the possible parasitic nature of the initial interaction, and the selection that guided the host to develop mechanisms to convert any initial detrimental effects of the symbiosis to beneficial or at least neutral effects (Blackstone 1995). In a resting state, mitochondria are known to release superoxide radicals, O2-. These molecules attack flavoproteins and carbon-carbon double bonds, destroying cell membranes as well as mechanisms for cell replication (Blackstone and Green 1999).
If this occurred in the protomitochondrion, there must have been a mechanism in place to stop it from killing its host cell, otherwise the symbiosis would never have continued. A possible solution is that the protomitochondrion may have used its aerobic metabolism to its advantage, and in doing so, the host cell was forced to protect itself. For example, the greater the amount of ATP generated by the symbiont for the host, the more energy the host would have to carry out cell division, giving the protomitochondrion the chance to “infect” other cells.
If the host cell could maintain a rapid rate of cell division, it could keep ATP in high demand. When modern mitochondria are exhibiting maximum phosphorylation to create large amounts of ATP, they generate very small amounts of the harmful superoxide radicals (Blackstone 1995). Thus, if the host could increase the rate at which it divided using the large amounts of symbiont-created ATP, it would decrease the chances that it could be damaged by the radicals, and the symbiont would now have several new hosts to infect, an ideal situation for both partners.
When organisms have been acquired by symbiosis, they can only be retained by their hosts if, when the host divides, a copy of the endosymbiont genome is passed to each daughter cell (Margulis 1981). One common method of retaining the endosymbionts in a host is by creating multiple copies of the endosymbiont so that there is a greater chance one of them will be passed on to each host generation (Margulis 1978). Adding to this idea, modern mitochondria have evolved the ability to divide synchronously with, though independent from, cell division so that there can be a sufficient number of them to be passed onto daughter cells (Gray et al. 999).
A second method for passing mitochondria to other generations is by packaging them in sperm cells, seeds, spores, and other reproductive cells (Margulis 1978). This must have evolved when sexual reproduction using cells such as gametes became prevalent in eukaryotes. Both of these mechanisms must have been important throughout the evolution of the mitochondria in cells, since host nuclei lack the genetic potential for forming new mitochondria (Lang et al. 1999). If these or similar events had not taken place, the protomitochondrion would not have continued past the first host cell.
Transition from Endosymbiont to Organelle The relationship between the current eukaryotic cell and its mitochondria is now entirely obligate, that is, one cannot survive without the presence of the other in aerobic environments (Rotte et al. 2000). Therefore, there must have been several changes in the endosymbiotic bacteria that became the mitochondria observed today. One of the first modifications to occur to the protomitochondria would have been the loss of their cell walls.
Though required for protection while the bacteria were free-living, cell walls would have been dispensable in the controlled, osmotically regulated, buffered environment of the host’s cytoplasm, and would have been selected against (Margulis 1981). It can also be assumed that the loss of cell walls would aid in the transport of ATP and nutrients between the endosymbionts and the host cell. Secondly, the cristae, infoldings of the inner membrane of the mitochondrion, must also have been formed due to selective pressures (Becker et al. 2003).
Cristae differentiated in order to increase the surface area for the oxidative enzymes required for oxidation-reduction reactions, which would in turn increase the productivity of the organelle (Martin et al. 2001). When examining the genome of Rickettsia prowazekii, the most probable ancestor of the protomitochondrion, researchers found 876 genes, whereas a typical animal mitochondrion codes only about 37 genes (Sengbusch 2002). Thus, in the transition from being a fully independent bacterium to becoming an organelle, the mitochondrion must have “lost” some its genes.
There are three pathways that explain the disappearance of certain genes from the mitochondrial genome. Firstly, genes whose function became redundant or obsolete for a specialized organelle, such as those involved in nucleotide, lipid, and amino acid synthesis, would have been selected against, since the host cell could provide all necessary components for basic metabolic functions (Lang et al 1999). A second possible scenario to explain mitochondrial gene loss is elimination of a redundant gene followed by takeover of the corresponding function by nuclear genes (Lang et al 1999).
For example, in the ciliate protozoan Tetrahymena pyriformis, the mitochondrial DNA codes for only seven distinct tRNA genes. To ensure that all codons of mitochondrial protein-coding genes are translated properly, the missing tRNAs are imported from the cytosol (Lang et al. 1999). Since no similar tRNA genes are found in aerobic prokaryotes, the missing mitochondrial genes must have been replaced by genes in the nucleus, a process that was repeated several times during mitochondrial evolution. A third possibility by which genes disappeared from mitochondrial DNA is via migration of the mitochondrial genes to the nucleus (Martin 2000-2).
This is common of genes coding for subunits of enzyme complexes such as cytochrome C, protein components of the mitochondrial ribosome, and the ftsZ gene for cell division (Margulis 1981). The transfer of genes from one organism to another is a plausible explanation, but there are hurdles that would have to be crossed before the two genomes became fully integrated. If a mitochondrial gene were to transfer, it could be possible that the host may see it as an intruder as it would a virus and destroy it, or that the gene may not function anywhere but in the mitochondrion itself.
In order for a transferred gene to produce a product that functions in the mitochondria, it would first have to integrate, and then gain the necessary regulatory signals for gene expression and intracellular targeting (Fairbanks and Anderson 1999). Nevertheless, a stable chimeric nucleus (one containing both host and symbiont genes) was formed and is observed in eukaryotic cells today (Blackstone 1995). One still puzzling fact is that our nuclear genome contains scattered fragments of inactive mitochondrial genes, remnants of evolutionary pressures continuing to push toward a tighter integration of the two partners (Margulis 1981).
Perhaps the final product-complete loss of mitochondrial DNA-has not yet been attained. The Hydrogen Hypothesis The traditional endosymbiotic theory as presented above has been the common belief of most researchers for the past thirty years. More recently, however, a new hypothesis, named the hydrogen hypothesis by its creator William Martin (2000-1), has raised questions about the identities of the original endosymbiont and host, and also their initial interaction. The theory is based on new evidence of the ancestry of an organelle called the hydrogenosome.
This organelle is found in places such as the ciliate protist Nyctotherus ovalis, which lives in the oxygen-poor environment of cockroach intestines (Embley and Martin 1998). Hydrogenosomes do not use oxygen as an electron acceptor as mitochondria do, but anaerobically break down pyruvate by transferring electrons directly onto protons, producing ATP, hydrogen, and carbon dioxide. (Embley and Martin 1998).
These rod-like organisms resemble mitochondria, but many lack their own DNA, and with their odd metabolism, they were at one time given a reputation of being an “oddball organelle” (Rotte et al. 000). It was once believed that mitochondria and hydrogenosomes arose through different symbioses, one an anaerobic bacterium, the other an aerobic bacterium (Travis 1998). Recent research using anaerobic eukaryotic trichonomads, flagellated protists with hydrogenosomes, has shown that these organisms possess genetic material resembling that used by the mitochondria of aerobic cells (Travis 1998).
This new evidence pushes the mitochondria and hydrogenosomes towards a common ancestor. In order to be an ancestor for both organelles, it must have been a facultatively anaerobic ? proteobacterium that was able to synthesize ATP either through the oxygen-consuming electron transport chain of mitochondria, or through hydrogen-producing fermentation of hydrogenosomes, depending on its particular environment (Martin 2000-1). Then the question of why any host cell would ever establish a symbiotic relationship with such a bacterium must be addressed.
The current endosymbiotic theory suggests that the host was an anaerobic protoeukaryote that used an aerobic bacterium to generate more ATP (Travis 1998). William Martin et al. 2001) suggest that the host was actually an archaeon that was unable to metabolize organic molecules on its own, surviving on non-biological sources of hydrogen, carbon dioxide, and acetate. Establishing a close relationship with a proteobacterium that gives off all three as a waste product of metabolism would have given the archaeon the advantage of another food supply. The well-fed archaeon would grow to depend on its symbiont, and may have engulfed it in attempt to increase the amount of food that it was receiving (Travis 1998).
The host would then have to learn to feed its endosymbiont by stealing some of its genes for information, leaving the endosymbiont to be completely dependent on its host for food (Travis 1998). When the oxygen concentration in the host’s environment became greater, the endosymbiont could have switched to the more efficient aerobic means of ATP production, setting it on the road to becoming a mitochondrion (Martin et al 2001). The hosts that remained in an anaerobic environment would have endosymbionts that shed the aerobic metabolism and became hydrogenosomes (Martin et al 2001).
This is in fact an acceptable hypothesis, since the few known eukaryotes with hydrogenosomes live in environments with very little oxygen. The hydrogen hypothesis is gaining support due to significant molecular evidence, but it is still a rather new theory, not without its weaknesses. For example, the theory requires a large changeover in the metabolism of the host from an autotroph (making its ATP from non-organic fuel) to a heterotroph (generating energy from organic molecules), while the traditional endosymbiotic theory is a less dramatic, more gradual approach to the idea of mitochondrial evolution.
Martin mentions that the process in question is simply the reverse of when photosynthesis developed in plants, a concept that is not thought to be overly drastic (Martin 2000-1). Genetic sequencing has yet to test this hypothesis completely, but even if it does, reconstructing past events is extremely difficult and some ambiguous answers may remain. Conclusions Though the theories presented above have concrete evidence to support them, they are not intended to be the definitive answer to the question of mitochondrial origins.
Instead, they are dynamic hypotheses that should be supplemented with ideas of researchers from various backgrounds. Each theory has its own flaws, which can be expected, since they predict the nature of a biological interaction that began a billion or more years ago. It seems that the hydrogen hypothesis is gaining favour in the eyes of some researchers because of the molecular evidence and the possible identity of the host, but it still fails to identify any living ancestor of the facultatively anaerobic symbiont.
The endosymbiotic theory is still the most widely used, since it gives a more comprehensive, plausible explanation, but it lacks a well-defined host. Though I find the hydrogen hypothesis to be a fascinating explanation, the traditional endosymbiotic theory has been more successfully documented in scientific literature, and seems to be very promising. K. W. Jeon’s experiments alone show how a parasitic relationship can become an obligate symbiosis, an event which I think to be very similar to the acquisition of the protomitochondrion.
Mitochondrial genome analysis might be the key to determining how and when endosymbionts became functional organelles. It may also show that one of the two main theories is correct, or that neither are possible. The fact of the matter remains that although scientific advances are made every day, we will never be able to determine the exact sequence of events that led to the formation of our mitochondria. We can only speculate on these events since we cannot go back in time to observe them.