Physiological Perception Filters
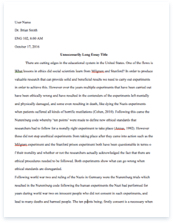
- Pages: 22
- Word count: 5332
- Category: Brain Perception
A limited time offer! Get a custom sample essay written according to your requirements urgent 3h delivery guaranteed
Order Now“When a person glimpses the face of a famous actor, sniffs a favourite food or hears the voice of a friend, recognition is instant. Within a fraction of a second after the eyes, nose, ears, tongue or skin is stimulated, one knows the object is familiar and whether it is desirable or dangerous. How does such recognition, which psychologists call preattentive perception, happen so accurately and quickly, even when the stimuli are complex and the context in which they arise varies?
Much is known about the way the cerebral cortex, the outer rind of the brain, initially analyses sensory messages. Yet investigations are only now beginning to suggest how the brain moves beyond the mere extraction of features-how it combines sensory messages with past experience and with expectation to identify both the stimulus and its particular meaning to the individual.
My own group’s studies, carried out over more than 30 years at the University of California at Berkeley, suggest that perception cannot be understood solely by examining properties of individual neurons, a microscopic approach that currently dominates neuroscience research. We have found that perception depends on the simultaneous, cooperative activity of millions of neurons spread throughout expanses of the cortex. Such global activity can be identified, measured and explained only if one adopts a macroscopic view alongside the microscopic one.
There is an analogy to this approach in music. To grasp the beauty in a choral piece, it is not enough to listen to the individual singers sequentially. One must hear the performers together, as they modulate their voices and timing in response to one another.
Our studies have led us as well to the discovery in the brain of chaos- complex behaviour that seems random but actually has some hidden order. The chaos is evident in the tendency of vast collections of neurons to shift abruptly and simultaneously from one complex activity pattern to another in response to the smallest of inputs.
This changeability is a prime characteristic of many chaotic systems. It is not harmful in the brain. In fact, we propose it is the very property that makes perception possible. We also speculate that chaos underlies the ability of the brain to respond flexibly to the outside world and to generate novel activity patterns, including those that are experienced as fresh ideas.
An understanding of perception must be based on knowledge of the properties of the neurons that enact it. My colleagues and I have concentrated in many of our studies on neurons of the olfactory system.
For years it has been known that when an animal or a person sniffs an odorant, molecules carrying the scent are captured by a few of the immense number of receptor neurons in the nasal passages; the receptors are somewhat specialized in the kinds of odorants to which they respond. Cells that become excited fire action potentials, or pulses, which propagate through projections called axons to a part of the cortex known as the olfactory bulb.
The number of activated receptors indicates the intensity of the stimulus, and their location in the nose conveys the nature of the scent. That is, each scent is expressed by a spatial pattern of receptor activity, which in turn is transmitted to the bulb.
The bulb analyses each input pattern and then synthesizes its own message, which it transmits via axons to another part of the olfactory system, the olfactory cortex. From there, new signals are sent to many parts of the brain-not the least of which is an area called the entorhinal cortex, where the signals are combined with those from other sensory systems. The result is a meaning-laden perception, a gestalt that is unique to each individual. For a dog, the recognition of the scent of a fox may carry the memory of food and expectation of a meal. For a rabbit, the same scent may arouse memories of chase and fear of attack.
Such knowledge has provided a valuable starting point for more detailed study of olfaction. However, it leaves two important issues unresolved. The first is the classic problem of separating foreground from background: How does the brain distinguish one scent from all others that accompany it?
“PHASE PORTRAITS” made from electroencephalograms (EEGs) generated by a computer model of the brain reflect the overall activity of the olfactory system at rest (above) and during perception of a familiar scent (right). Resemblance of the portraits to irregularly shaped, but still structured, coils of wire reveals that brain activity in both conditions is chaotic: complex but having some underlying order. The more circular shape of the right-hand image, together with its greater segregation of colour, indicates that olfactory EEGs are more ordered-more nearly periodic-during perception than during rest.
Also, how does the brain achieve what is called generalization-over- equivalent receptors? Because of turbulence in nasal airflow, only a few of the many receptors that are sensitive to an odorant are excited during a sniff, and the selection varies unpredictably from one sniff to the next. How does the brain recognize that signals from different collections of receptors all refer to the same stimulus? Our investigations begin to suggest answers to both problems.
Many of our insights were derived from intensive studies of the olfactory bulb. Those experiments show clearly that every neuron in the bulb participates in generating each olfactory perception. In other words, the salient information about the stimulus is carried in some distinctive pattern of bulbwide activity, not in a small subset of feature-detecting neurons that are excited only by, say, and foxlike scents.
Moreover, although this collective neural activity reflects the odorant, the activity itself is not determined solely by the stimulus. Bulbar functioning is self-organized, very much controlled by internal factors, including the sensitivity of the neurons to input.
The experiments uncovering the collective activity were conceptually simple. By applying standard reinforcement techniques, we trained animals, often rabbits, to recognize several different odorants and to behave in particular ways when they did-for instance, to lick or chew in expectation of food or water. Before training was started, we attached 60 to 64 electrodes 0.5 millimetre apart in a gridlike array to a large part of the bulbar surface.
During training and thereafter, the array enabled us to collect sets of 60 to 64 simultaneously recorded electroencephalogram (EEG) tracings as the animals breathed in and out, sometimes sniffing familiar scents and sometimes not. Each tracing reflects the mean excitatory state of local pools of neurons lying in a well-defined layer immediately beneath the electrodes. Rises in the wavelike tracings indicate increasing excitement; dips represent diminished excitement caused by inhibition.
The EEGs should not be confused with recordings of impulses fired by individual axons or by pools of neurons, although each EEG is related to the firing pattern of neurons in a neighbourhood of the cerebral cortex. The tracings detect essentially the same information that neurons assess when they “decide” whether or not to fire impulses, but an EEG records that information for thousands of cells at once.
To better understand exactly what the EEG shows, it helps to know some of the details of how cortical neurons operate. Such cells continuously receive pulses-usually at projections known as dendrites-from thousands of other neurons. The pulses are conveyed at specialized junctions called synapses. Certain incoming pulses generate excitatory waves of electric current in the recipients; others generate inhibitory waves [see top illustration on page 82]. These currents-“dendritic currents”-are fed through the cell body (which contains the nucleus) to a region called the trigger zone, at the start of the axon.
There the currents cross the cell membrane into the extracellular space. As they do, the cell calculates the overall strength of the currents (reflected in changes in voltage across the membrane), essentially by adding excitatory currents and subtracting inhibitory ones. If the sum is above a threshold level of excitation, the neuron fires.
The mechanism producing each EEG tracing similarly sums the currents initiated at the dendrites, but it taps the currents after they leave the cell. The tracings reflect the excitatory state of groups of neurons rather than of individual ones, because the extracellular space is traversed by currents from thousands of cells.
BASIC FLOW OF OLFACTORY INFORMATION IN THE BRAIN
In our experiments, the EEG tracings from the electrodes in an array are as unpredictable and irregular as freehand scrawls. Yet they manifest perceptual information.
In living individuals, EEGs always oscillate, or rise and fall, to some extent, but the oscillations are usually quite irregular. When an animal inhales a familiar scent, what we call a burst can be seen in each EEG tracing. All the waves from the array of electrodes suddenly become more regular, or ordered, for a few cycles-until the animal exhales. The waves often have a higher amplitude (height) and frequency than they do at other times.
The burst waves are often called 40-hertz waves, meaning that they oscillate at about 40 cycles per second. Because the frequency can actually range from 20 to 90 hertz, I prefer to call them gamma waves, in analogy with a range of high frequency X rays.
The fact that the bursts represent cooperative, interactive activity is not immediately clear in the EEG plots, because the burst segments differ in shape from tracing to tracing in a simultaneously recorded set. Nevertheless, by taxing our computers, we find we are able to tease out evidence of collective behaviour from the complex background. In each set of burst recordings, we can identify a common waveform, or carrier wave: a shared pattern of rises and falls that is embedded in each tracing. The average amplitude is not identical across the set-some versions of the carrier wave are shallow, and others are deep. However, all of them curve up and down nearly in synchrony. The common behaviour makes up between one quarter and three quarters of the total activity of the neurons giving rise to each trace.
Curiously, it is not the shape of the carrier wave that reveals the identity of an odour. Indeed, the wave changes every time an animal inhales, even when the same odorant is repeatedly sniffed. The identity of an odorant is reliably discernible only in the bulbwide spatial pattern of the carrier- wave amplitude [see top illustration on page 84].
Amplitude patterns become especially clear when we plot the average amplitude of the individual versions of the carrier wave on a grid representing the surface of the bulb. The resulting “maps” resemble contour diagrams that indicate the elevations of mountains and valleys. As long as we do not alter the animals’ training, the same map emerges every time an animal sniffs a particular odorant, even though the carrier wave differs with each sniff.
These maps have helped demonstrate not only that perception requires global bulbwide activity but also that the bulb participates in assigning meaning to stimuli. The amplitude map representing a given odorant changes strikingly when we alter the reinforcement associated with that scent. If the bulb did not bring experience to bear on perception, the map would remain constant even after the conditioned association had been changed.
NEURONS OF THE OLFACTORY SYSTEM share information through a rich web of synapses, junctions where signals flow from neuron to neuron. Usually signals pass from projections called axons to projections called dendrites, but sometimes they pass from dendrite to dendrite or axon to axon. The widespread sharing leads to collective activity. In this highly schematic diagram, red shading signifies that a neuron is exciting another cell, black shading that a neuron is inhibiting another.
We believe that something we call the nerve cell assembly is both a crucial repository of past associations and an essential participant in the formation of the collective bulbar burst. The hypothetical assembly consists of neurons that have simultaneously been excited by other neurons during learning.
More than 20 years ago, my colleagues and I discovered that when animals are trained by reinforcement techniques to discriminate olfactory stimuli, certain synapses that connect neurons within the bulb and within the olfactory cortex become selectively strengthened during the training. That is, the sensitivity of the postsynaptic cells to excitatory input-a property known as gain-is increased at the synapse, so that an input generates a greater dendritic current than it would have generated in the absence of special training. Technically, gain is the ratio of output to input-here, the net strength of the dendritic currents to the number of incoming pulses. The strengthening occurs not in the synapse between an input axon (such as a receptor from the nose) and the neuron it excites (such as a bulbar neuron) but in the synapse between connected neurons that are simultaneously excited by input neurons during learning. Neurons in the bulb and in the olfactory cortex are connected to many others in those regions.
EEG WAVES reflect the mean excitation of pools of neurons. Excitatory inputs at synapses generate electric currents that flow in closed loops within the recipient neuron toward its axon, across the cell membrane into the extracellular space and, in that space, back to the synapse (red arrows). Inhibitory inputs generate loops moving in the opposite direction (black arrows). In cells the trigger zone adds current strengths (reflected in changes in voltage across the membrane), and it fires impulses if the sum is sufficiently positive. Electrodes on the brain tap those same currents after they leave the cell. The resulting EEGs indicate the excitation of whole groups of cells, not individuals, because the extracellular avenues from which the EEGs arise carry currents contributed by thousands of cells.
Such strengthening is predicted by the widely accepted Hebb rule, which holds that synapses between neurons that fire together become stronger, as long as the synchronous firing is accompanied by a reward. (The strengthening is now known to involve “modulator” chemicals that the brain stem releases into the bulb and cortex during reinforcement.) We infer from our data that a nerve cell assembly, consisting of neurons joined by Hebbian synapses, forms for a particular scent as an individual is reinforced for learning to identify that odorant. Thereafter, when any subset of neurons in the assembly receives the familiar input, the entire assembly can rapidly become stimulated, as excitatory signals speed across the favoured Hebbian synapses. The assembly, in turn, directs the rest of the bulb into a distinct pattern of activity.
If we are correct, the existence of a nerve cell assembly would help explain both the foreground-background problem and generalization-over- equivalent receptors. In the first instance, the assembly would confer “frontrunner” status on stimuli that experience, stored in the Hebbian synapses has made important to the individual. In the second instance, the assembly would ensure that information from any subset of receptors, regardless of where in the nose they were located, would spread immediately over the entire assembly and from there to the rest of the bulb.
SIMULTANEOUS RECORDINGS from the olfactory bulb (a) and front (b) and rear (c) parts of a cat’s olfactory cortex show low-frequency waves interrupted by “bursts”-high-amplitude, high-frequency oscillations that are generated when odours are perceived. The average amplitude of a burst is some 100 microvolts. Each lasts a fraction of a second, for the interval between inhalation and exhalation.
As important as the nerve cell assembly is to perception, it does not by itself generate bulbwide bursts of collective activity. For a burst to occur in response to some odorant, the neurons of the assembly and the bulb as a whole must first be “primed” to respond strongly to input.
Two important processes complement the priming accomplished by the development of Hebbian synapses. Both processes affect the gain, doing so by altering the sensitivity of the trigger zones, not the synapses. Here the gain is the ratio of the number of pulses fired (output) to the net dendritic current (input). The total gain is the product of the gain at the synapses and trigger zones.
One primer is general arousal. Our experiments show that the gain in neuronal collectives increases in the bulb and olfactory cortex when an animal is hungry, thirsty, sexually aroused or threatened [see illustration on page 85]. Such priming seems to be accomplished by axons from elsewhere in the brain that release modulatory chemicals (other than those involved in forming Hebbian synapses).
The other primer is input itself. When cortical neurons are excited, their output increases. Each new input they receive while they are still excited raises their output markedly, indicating that their gain has been increased by the input. This increase occurs over a particular range of input. If the net input is strongly inhibitory, no pulses are fired. Above some very high level of excitatory input, neurons fire at their maximal rate and cannot do more, even if the input is increased. In the wide range between, however, pulse output increases along a sigmoid (S-shaped) curve. The steepness, or slope, of the curve reflects the gain.
The discovery of an increase in gain with excitation is particularly noteworthy because most neural network models assume neurons are at maximum gain when they are at rest. Both excitation and inhibition are generally assumed to decrease gain, so that the networks constantly maintain stability. Such assumptions are inappropriate for the brain because they do not allow networks to generate explosive changes. Hence, it seems that information from odorants is fed by a small number of receptors to a still smaller number of cells in the bulb. If the odorant is familiar and the bulb has been primed by arousal, the information spreads like a flash fire through the nerve cell assembly. First, excitatory input to one part of the assembly during a sniff excites the other parts, via the Hebbian synapses. Then those parts reexcite the first, increasing the gain, and so forth, so that the input rapidly ignites an explosion of collective activity throughout the assembly. The activity of the assembly, in turn, spreads to the entire bulb, igniting a full-blown burst.
The bulb then sends a “consensus statement” simultaneously along parallel axons to the olfactory cortex. What must next be made clear is how that cortical area distinguishes the consensus statement from the background of other stimuli impinging on it from the bulb and elsewhere.
WHY EEG WAVES OSCILLATE
Alternating rises and falls in amplitude stem from negative-feedback circuits that are established by the interaction of pools of excitatory and inhibitory neurons. When the pools have been sensitized to input, even a small input can trigger. A burst of high-amplitude oscillation. The diagrams represent neuronal activity at the end of each quarter cycle. Dark shading signifies great excitement; lighter shading signifies less excitement.
The answer undoubtedly has to do with the wiring joining the bulb to the cortex. The bulb generates trains of impulses that run simultaneously along the parallel axons leading from the bulb to the cortex. Each axon branches extensively and transmits pulses to many thousands of neurons across the olfactory cortex, and each cortical target cell receives input from thousands of bulbar cells.
The carrier activity of the incoming lines, which is synchronized by cooperation, probably stands out for the simple reason that such signals add together- nonsynchronous inputs, which are not at the carrier frequency and phase, effectively cancel one another. Thus, every recipient neuron in the olfactory cortex picks up a share of the cooperative bulbar signal and transmits the summed signals to thousands of its neighbours simultaneously.
In response, the massively connected neurons of the cortex, which have formed their own nerve cell assemblies, promptly generate their own collective burst, albeit one having a carrier wave and a spatial amplitude pattern that differ from those in the bulb. In essence, the transmission pathway for the global pattern in the bulb launders the bulbar message; it removes “noise,” so that only the collective signal affects the olfactory cortex significantly. Just as a burst in the bulb guarantees the delivery of a coherent message to the cortex, so presumably does the global burst in the cortex enable outgoing messages from that region to stand above the din when they reach other regions of the brain.
There are many reasons why we believe the activity of the brain both during and between bursts is chaotic, not merely random. However, before I delve into those reasons, let me clarify further, what is meant by chaos. At the risk of oversimplification, I sometimes like to suggest the difference between chaos and randomness by comparing the behaviour of commuters dashing through a train station at rush hour with the behaviour of a large, terrified crowd. The activity of the commuters resembles chaos in that although an observer unfamiliar with train stations might think people were running in many directions without reason, order does underlie the surface complexity: everyone is hurrying to catch a specific train. The traffic flow could rapidly be changed simply by announcing a track change. In contrast, mass hysteria is random. No simple announcement would make a large mob become cooperative.
One of the most convincing early clues to the presence of chaos was an aperiodic (nonrepeating) common carrier wave everywhere in the bulb not only during bursts but also between bursts-even when there was no extrabulbar stimulus driving that collective activity. The lack of external driving meant the activity was self-generated by the bulb. Such self- organization is a characteristic of chaotic systems [see “Chaos,” by James B. Crutchfield, J. Doyne Farmer, Norman H. Packard and Robert S. Shawl SCENTIFIC AMERICAN, December 1986].
Another clue was the apparent ability of neural collectives in the bulb and cortex to jump globally and almost instantly from a nonburst to a burst state and then back again. Rapid state changes are called phase transitions by physicists and bifurcations by mathematicians. Whatever they are called, dramatic changes in response to weak input are, it will be recalled, another feature of chaotic systems. Bifurcation is significantly harder to control in random systems.
We gained more evidence for chaos by developing computer models of the olfactory system as a whole: the bulb, the cortex, the connections between them and the input to both areas from outside the system. We simulated the activity of the system by solving sets of ordinary differential equations that describe the dynamics of local pools of neurons.
First, we demonstrated that the model did in fact represent the olfactory system accurately. With no more than a single pulse (equivalent to excitation of a few receptors) to start the system, the model sustained activity that closely resembled aperiodic olfactory EEGs.
After we ” trained” the model to recognize specific odorants, the bulbar segment generated bursts in response to the selected inputs, and the embedded common carrier waves yielded distinct and consistent amplitude maps. Moreover, whenever we added a new “odorant” to the perceptual repertoire of our hypothetical subject, an identifying global amplitude map was created. At the same time, the other maps changed-as they should, of course, in a true associative memory system. We had earlier found such changes in test animals after they were trained to recognize stimuli beyond the ones they had learned initially.
COMMON CARRIER WAVE emerged from 60 EEGs recorded information is contained in the spatial pattern of amplitude simultaneously from the olfactory cortex of a rabbit as it recognized a scent (left). The wave is nearly the same in each recording, except that the amplitude varies. The shape of the carrier wave does not indicate the identity of the scent. That information is contained in the spatial pattern of amplitude across the cortex, which can be displayed as a contour plots(right), much like the plots of elevations in topographic maps. The coloured contours represent the highest amplitude; successive contours represent the lower amplitudes.
Our model yielded additional evidence for chaos when we coaxed it to produce mock EEGs of extended bursts and of “interburst” activity in the intervals between bursts. Because the artificial EEGs persisted longer than EEGs normally do, we were able to plot what are called phase portraits of the predicted behaviour of the olfactory system, both during and between bursts. The portraits can show at a glance whether the dynamics may be chaotic.
The details of how such portraits are made and why they are a valid reflection of global activity in the olfactory system are too complex to discuss at length. Nevertheless, for those readers familiar with phase portraits, I should note that we plotted the portraits in a three- dimensional grid and added colour to display a fourth dimension. Each axis represented EEG amplitude from some part of the olfactory system, such as the bulb or a subsection of the olfactory cortex. A range of colours from red to blue represented high to low amplitude from a fourth part of the system.
We plotted as a point one set of three amplitudes, measured at a given moment. Next, we plotted another point from the set, representing a thousandth of a second later, and connected the two points with a coloured line. Then we plotted a third point, and so on. We rotated the final image in space to find the most informative point of view.
CONTOUR PLOT at the left emerged consistently from bulbar EEG’s of a rabbit that had been conditioned to associated the scent of sawdust with a particular reinforcement. After the animal learned to recognize the smell of banana (middle), however, reexposure to sawdust led to the emergence of a new sawdust plot (right). The change shows that bulbar activity is dominated more by experience than by stimuli; otherwise, sawdust would always give rise to the same plot.
The pictures supported the possibility of chaos, because the images resembled loose coils of wire having different shapes and colour distributions. If the model olfactory system had behaved randomly, there would be no coherent shapes, just dots spread everywhere, like “snow” on a television set. If, on the other hand, the system was predictable in detail, the shapes would be simpler; they might resemble a spiral, a folded circle or a torus (a doughnut).
The shapes we found represent chaotic attractors. Each attractor is the behaviour the system settles into when it is held under the influence of a particular input, such as a familiar odorant. The images suggest that an act of perception consists of an explosive leap of the dynamic system from the “basin” of one chaotic attractor to another; the basin of an attractor is the set of initial conditions from which the system goes into a particular behaviour. The bottom of a bowl would be a basin of attraction for a ball placed anywhere along the sides of the bowl. In our experiments, the basin for each attractor would be defined by the receptor neurons that were activated during training to form the nerve cell assembly.
We think the olfactory bulb and cortex maintain many chaotic attractors, one for each odorant an animal or human being can discriminate. Whenever an odorant becomes meaningful in some way, another attractor is added, and all the others undergo slight modification.
SIGMOID CURVES show the relation between input (wave density) and output (pulse density) at trigger zones in populations of neurons. (The plots are not valid for individual neurons.) The rising steepness associated with increased arousal indicates that sensitivity to input-or gain (the ratio of output to input, or the slope)-rises with arousal. In each case, gain also increases as neurons that are already excited (those at and to the right of the circles) receive more stimulation. This input-dependent increase in gain is essential to the formation of bursts
Identification of chaos does not automatically reveal its source. We suspect chaos in the brain arises when two or more areas of the brain, such as the bulb and the olfactory cortex, meet at least two conditions: they excite one another strongly enough to prevent any single part from settling down, and, at the same time, they are unable to agree on a common frequency of oscillation. Competition between the parts would increase the sensitivity and instability of the system contributing to chaos. Part of the evidence for the importance of interaction between the bulb and the cortex is that disconnection of the two regions makes chaos disappear; both parts become abnormally stable and quiet.
Modulatory chemicals released into the system from elsewhere in the brain also increase sensitivity to input, both by participating in the formation of the Hebbian synapses in nerve cell assemblies and by enhancing arousal. Because various factors maintain great sensitivity, a very small signal-a whiff, a whisper, a glimpse-can trigger a massive, collective state change.
Conceivably, the chaos we have observed is simply an inevitable by- product of the brain’s complexity, including its myriad connections. Yet our evidence suggests that the controlled chaos of the brain is more than an accidental by-product. Indeed, it may be the chief property that makes the brain different from an artificial-intelligence machine.
One profound advantage chaos may confer on the brain is that chaotic systems continually produce novel activity patterns. We propose that such patterns are crucial to the development of nerve cell assemblies that differ from established assemblies. More generally, the ability to create activity patterns I may underlie the brain’s ability to generate insight and the “trials” of trial and-error problem solving.
We have found widespread, apparently chaotic behaviour in other parts of the brain. That finding does not necessarily imply that other sensory systems operate as the olfactory system does. However, we think they do. Indeed, we and other investigators have documented gamma bursts across large cortical regions involved in recognizing visual images. As in the olfactory system, familiar visual stimuli are associated with specific amplitude maps of common carrier waves. I predict that when people examine drawings in which foreground and background are ambiguous, so that perception alternates between two images, the amplitude maps will be found to alternate as well.
I begin to envision the general dynamics of perception. The brain seeks information, mainly by directing an individual to look, listen and sniff. The search results from self-organizing activity in the limbic system (a part of the brain that includes the entorhinal cortex and is thought to be involved in emotion and memory), which funnels a search command to the motor systems. As the motor command is transmitted, the limbic system issues what is called a reafference message, alerting all the sensory systems to prepare to respond to new information.
Moreover, respond they do, with every neuron in a given region participating in a collective activity-a burst. Synchronous activity in each system is then transmitted back to the limbic system, where it combines with similarly generated output from other sensory systems to form a gestalt. Then, within a fraction of a second, another search for information is demanded, and the sensory systems are prepared again by reafference.
Consciousness may well be the subjective experience of this recursive process of motor command, reafference and perception. If so, it enables the brain to plan and prepare for each subsequent action on the basis of past action, sensory input and perceptual synthesis. In short, an act of perception is not the copying of an incoming stimulus. It is a step in a trajectory by which brains grow, reorganize themselves and reach into their environment to change it to their own advantage.
The poet William Blake wrote: “If the doors of perception were cleansed every thing would appear to man as it is, infinite.” Such cleansing would not be desirable. Without the protection of the doors of perception-that is, without the self-controlled chaotic activity of the cortex, from which perceptions spring-people and animals would be overwhelmed by eternity.” – Copyright 1991: Walter J. Freeman