Biosensors Nano
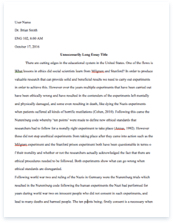
- Pages: 14
- Word count: 3291
- Category: Biochemistry
A limited time offer! Get a custom sample essay written according to your requirements urgent 3h delivery guaranteed
Order NowIntroduction:
Over the past three decades we have witnessed a tremendous amount of activity in the area of biosensors. Biosensors are small devices employing biochemical molecular recognition properties as the basis for a selective analysis. The major processes involved in any biosensor system are analyte recognition, signal transduction, and readout. Due to their specificity, speed, portability, and low cost, biosensors offer exciting opportunities for numerous decentralized clinical applications. Electrochemical devices have traditionally received the major share of the attention in biosensor development. Such devices produce a simple, inexpensive and yet accurate and sensitive platform. The name electrochemical biosensor is applied to a molecular sensing device which intimately couples a biological recognition element to an electrode transducer. The purpose of the transducer is to convert the biological recognition event into a useful electrical signal.
Amperometric and potentiometric transducers are most commonly used in conjunction with electrochemical biosensors. In potentiometric devices, the analytical information is obtained by converting the biorecognition process into a potential signal in connection to the use of ion selective electrodes (ISE). Amperometric biosensors operate by applying a constant potential and monitoring the current associated with the reduction or oxidation of an electroactive species involved in the recognition process. An amperometric biosensor may be more attractive because of its high sensitivity and wide linear range. Elegant research on new sensing concepts, coupled with numerous technological innovations, has opened the door to widespread clinical applications of amperometric devices. The high sensitivity, specificity, simplicity, and inherent miniaturization of modern electrical bioassays permit them to rival the most advanced optical protocols.
Such miniaturization allows packing of numerous microscopic electrode transducers onto a small footprint of a biochip device, and hence the design of high-density arrays. Nanotechnology has recently become one of the most exciting forefront fields in analytical chemistry. Nanotechnology is defined as the creation of functional materials, devices and systems through control of matter at the 1–100 nm scale. A wide variety of nanoscale materials of different sizes, shapes and compositions are now available [1] The huge interest in nanomaterials is driven by their many desirable properties. In particular, the ability to tailor the size and structure and hence the properties of nanomaterials offers excellent prospects for designing novel sensing systems and enhancing the performance of the bioanalytical assay.
The unique properties of nanoscale materials offer excellent prospects for interfacing biological recognition events with electronic signal transduction and for designing a new generation of bioelectronic devices exhibiting novel functions. In the present paper, I have elaborated upon the recent developments in the application of nanomaterials to electrochemical biosensing,
Nanostructured materials:
Metal nanoparticles are generally defined as isolable particles between 1 and 50 nm in size, that are prevented from agglomerating by protecting shells. Owing to their small size such nanoparticles have physical, electronic and chemical properties that are different from those of bulk metals. Such properties strongly depend on the number and kind of atoms that make up the particle. Typically, such particles are prepared by chemical reduction of the corresponding transition metal salts in the presence of a stabilizer (capping agent such as citrate or thiol) which binds to their surface to impart high stability and rich linking chemistry and provide the desired charge and solubility properties. Designer particles, including colloidal gold or inorganic nanocrystals have found broad applications in many forms of biological tagging schemes.
For example, colloidal quantum dots have been widely used for optical bioassays because their light emitting properties can be broadly tuned through size variation [2]. One-dimensional (1-D) nanostructures, such as carbon nanotubes (CNT) and semiconductoror conducting polymer nanowires, are particularly attractive for bioelectronic detection. Because of the high surface-to-volume ratio and novel electron transport properties of these nanostructures, their electronic conductance is strongly influenced by minor surface perturbations (such as those associated with the binding of macromolecules).
Such 1-D materials thus offer the prospect of rapid (realtime) and sensitive label-free bioelectronics detection, and massive redundancy in nanosensor arrays. The extreme smallness of these nanomaterials would allow packing a huge number of sensing elements onto a small footprint of an array device. Metal and conducting polymer nanowires can be readily prepared by a template-directed electrochemical synthesis involving electrodeposition into the pores of a membrane template [3]. Carbon nanotubes (CNT) are particularly exciting 1-D nanomaterials that have generated a considerable interest owing to their unique structure-dependent electronic and mechanical properties [4]. CNT can be divided into single-wall carbon nanotubes (SWCNT) and multi-wall carbon-nanotubes (MWCNT).
SWCNT possess a cylindrical nanostructure (with a high aspect ratio), formed by rolling up a single graphite sheet into a tube. SWCNT can thus be viewed as molecular wires with every atom on the surface. MWCNT comprise of an array of such nanotubes that are concentrically nested like rings of a tree trunk. The remarkable properties of CNT suggest the possibility of developing superior electrochemical sensing devices, ranging from amperometric enzyme electrodes to label-free DNA hybridization biosensors. The tailored electronic conductivity of conducting polymers, coupled with their ease of processing/modification and rich chemistry, make them extremely attractive as 1-D sensing materials. Newly introduced CNT/ conducting-polymer nanowire materials, based on incorporating oxidized CNT as the charge-balancing dopants within electropolymerized wires, should further enhance the sensing capabilities of 1-D materials.
Electrochemical biosensors derived from nanostructured materials: 1. Nanomaterial based enzyme electrodes: Enzyme electrodes have been widely used for monitoring a wide range of clinically or environmentally important substrates. An extremely important challenge in amperometric enzyme electrodes is the establishment of satisfactory electrical communication between the active site of the enzyme and the electrode surface. [5] The redox center of most oxidoreductases is electrically insulated by a protein shell. Because of this shell, the enzyme cannot be oxidized or reduced at an electrode at any potential. The possibility of direct electron-transfer between enzymes and electrode surfaces could pave the way for superior reagentless biosensing devices, as it obviates the need for co-substrates or mediators and allows efficient transduction of the biorecognition event.
‘‘Trees’’ of aligned CNT in the nanoforest, prepared by self assembly can act as molecular wires to allow electrical communication between the underlying electrode and redox proteins. (covalently attached to the ends of the SWNT).[6,7] Willner’s group [8] demonstrated that aligned reconstituted glucose oxidase (GOx) on the edge of SWCNT can be linked to an electrode surface. Such enzyme reconstitution on the end of CNT represents an extremely efficient approach for ‘plugging’ an electrode into GOx. Electrons were thus Transported along distances higher than 150 nm with the length of the SWCNT controlling the rate of electron transport.
An interfacial electron transfer rate constant of was estimated for 50 nm long SWCNT. The catalytic properties of metal nanoparticles have also facilitated the electrical contact of redox centers of proteins with electrode surfaces. For example, gold nanoparticles were shown to be extremely useful as electron relays (‘‘electrical nanoplugs’’) for the alignment of glucose oxidase on conducting supports and wiring its redox center.[9] A wide range of enzyme electrodes based on dehydrogenase or oxidase enzymes rely on amperometric monitoring of the liberated NADH or hydrogen peroxide products. The anodic detection of these species at ordinary electrodes is often hampered by the large overvoltage encountered for their oxidation. The greatly enhanced redox activity of hydrogen peroxide[10] and NADH17 at CNT-modified electrodes addresses these overvoltage limitations and makes these nanomaterials extremely attractive for numerous oxidase- and dehydrogenasebased amperometric biosensors.
The ability of CNT to promote electron transfer reactions is attributed to the presence of edge plane defects at their end caps. Carbon-nanotube-modified electrodes have also been shown to be extremely useful for circumventing surface fouling associated with the oxidation of the liberated NADH product.[11] The deposition of platinum nanoparticles onto CNT has led to further improvements in the detection of the enzymatically-liberated peroxide species. [12] In addition to CNT films, it is possible to use CNT-based inks and pastes for designing screen-printed and biocomposite, respectively, amperometric biosensors. The excellent electrocatalytic properties of metal nanoparticles (compared to bulk metal electrodes) can also benefit amperometric enzyme electrodes.
For example, Niwa and coworkers[13] dispersed iridium nanoparticles (2 nm diameter) in graphite-like carbon and used the resulting transducer for improved amperometric biosensing of glutamate. Tao’s group[14] described a conducting polymer nanosensor for detecting glucose based on a pair of nanoelectrodes, separated with a small (20–60 nm) gap connected by a polyanaline/glucoseoxidase film. The remarkable small dimensions of the new device, coupled with its very fast response and minimal oxygen consumption, makes it attractive for in-vivo monitoring of glucose. Another promising and controllable route for preparing conducting-polymer nanowire enzyme sensors involves electrodeposition within the channel between Electrodes[15]
Fig a. Assembly of SWCNT electrically contacted glucose oxidase electrode: linking the reconstituted enzyme, on the edge of the FAD functionalized SWCNT, to the electrode surface.[41]
2. Electrochemical immunoassays and protein assays:
Abnormal concentrations of certain proteins can indicate the presence of various cancers. For the past two decades Heineman’s group has developed highly sensitive enzyme electrochemical immunoassays [16]. Such protocols rely on labeling of the antibody (or antigen) with an enzyme which acts on a substrate and generate an electroactive product that can be detected amperometrically. Enzyme immunosensors can employ competitive or sandwich modes of operation. In addition to enzyme labels, it is possible to use metal markers and redox tags for electronic transduction of antigen–antibody interactions.
The development of electrochemical immunosensors for measuring tumor markers was recently reviewed. [17]. The first electrochemical immunosensor for a cancer marker was developed in the late 1970s [18]. The device involved a competitive assay of hCG (human chorionic gonadotropin) in connection to a catalase label and amperometric monitoring of the enzymatic reaction. Meyerhoff’s group reported on a novel sandwich electrochemical enzyme immunoassay for the simultaneous measurement of the PSA (Prostate-Specific Antigen) and hCG protein markers in undiluted whole blood [19]. A gold-coated microporous membrane facilitated direct assays, without separation or washing steps. Such simplification holds great promise for the creation of portable point-of-care test systems.
Ju’s group reported on a reagentless amperometric immunosensor based on direct electrochemistry of the enzyme label horseradish peroxidase for measuring low levels of carcinoma antigen 125 [20]. Jin’s group described a capillary electrophoretic enzyme noncompetitive immunoassay with an electrochemical detection for measuring the CA125 tumor marker [21]. Microfluidic devices, carrying out such capillary-electrophoretic enzyme immunoassays with amperometric detection on microchip platforms [22], should be extremely attractive for future cancer diagnostics. The integration of additional (sample processing/manipulations) steps onto these ‘Lab-on- Chip’ devices should facilitate assays of complex biological fluids. It is well recognized now that simultaneous measurements of combination of tumor markers (i.e., distinct protein patterns) can play a major role in the diagnosis of cancer [23].
Protein signature patterns, reflecting different stages of the disease, can be readily detected using immunosensor chips based on multiple antibody-functionalized transducers. Electrode arrays, having multiple individually addressable electrochemical transducers, can be readily fabricated to meet the requirements of multi-analyte protein arrays. The use of a single enzyme label with such arrays requires proper spatial separation of the individual transducers to eliminate cross talk problems due to diffusion of the enzymatically generated electroactive species from one electrode to the neighboring one. For example, Karube’s group designed an electrochemical protein array consisting of 36 platinum working electrodes, with different capture antibodies, and operated in the sandwich enzyme immunoassay mode [24]. No apparent cross reactivity was observed using interelectrode distances of 700 um.
Wilson (2005) described recently a dualelectrode enzyme immunosensor for simultaneous amperometric measurements of the two tumor markers carcinoembryonic antigen (CEA) and fetoprotein (AFP). High sensitivity down to the 1 ng/mL level was reported, along with the absence of cross-talk effects. Nucleic acid ligands, known as aptamers, also offer great promise for electrochemical sensing of proteins. Label-free detection of aptamer–protein interactions has been documented recently in connection to electrochemical impedance spectroscopic transduction of the binding event [25]. One-dimensional (1D) nanostructures, such as semiconductor- or conducting-polymer nanowires (NW), are extremely attractive for designing high-density protein arrays.
Because of their high surface-to-volume ratio and novel electron transport properties, their electronic conductance is strongly influenced by minor surface perturbations (e.g., binding of biomolecules), hence indicating great promise for label-free real-time protein detection. Due to the extreme smallness of these nanomaterials, it is possible to pack a large number of antibody-functionalized NW onto a remarkably small footprint of an array device [26]. Such 1D materials thus offer the prospect of massive redundancy in nanosensor arrays and suggest great promise for assays of multiple disease markers in ultrasmall sample volumes.
Several studies already indicated the potential of functionalized NW for highly sensitive real-time biodetection. For example, Lieber’s laboratory demonstrated highly sensitive protocols for monitoring DNA hybridization [27] or single viruses [28] in connection to p-type silicon NW (SiNW) functionalized with PNA probes or antibodies for influenza, respectively. Discrete conductance changes, characteristic of the binding event, were observed at extremely low target concentrations. A contribution demonstrated the use of an antibody-functionalized silicon nanowire sensor array for the multiplexed label-free real-time monitoring of cancer markers in undiluted serum samples [29]. The biosensing utility of other 1D nanomaterials, such as conducting polymer NW or carbon-nanotubes, has also been illustrated [30,31]
Fig b. DNA hybridization measurements at SiNW functionalized with PNA probes. (A) Realtime conductance response to 60 fM WT DNA sample. The arrow marks the point in time when the sample was added. The inset shows a SEM image of a typical SiNW device with source (S) and a drain (D) indicated; scale bar is 1m. (B) Time dependent conductance in DNA-free solution; the arrow indicates the point in time when a new solution sample was added [42]
3. Nanoparticle based bioelectronics assays:
In addition to nanowires, it is possible to use nanoparticles tags for designing electrical bioaffinity assays with remarkable sensitivity and multiplexing capability. The rapid progress of nanomaterial-based electrochemical biosensors suggests the major impact they may have upon cancer diagnostics in the near future. The ultrahigh sensitivity of such nanoparticle-based electrochemical sensing protocols opens up the possibility of detecting cancer markers that cannot be measured by conventional methods and could lead to an early detection of the disease. Stripping voltammetry has been particularly useful for detecting metal nanoparticle tags, owing to its ‘built-in’ accumulation (electrodeposition) step that leads to a remarkable sensitivity [32].
Two groups [33,34] demonstrated the use of gold nanoparticle tracers for stripping-based electrochemical detection of DNA hybridization and antibody–antigen interactions. Such protocols relied on capturing the gold nanoparticles to the hybridized target or captured antigen, followed by their dissolution and electrochemical stripping measurement of the metal tracer. A triple-amplification bioassay, coupling polymeric carrier-sphere amplifying units (loaded with numerous gold nanoparticles tags) with the preconcentration feature of the electrochemical stripping detection and a catalytic electroless enlargement of the multiple gold-particle tags was also demonstrated [35]. Adramatic signal amplification can be achieved by linking the biorecognition units to polymeric microbeads carrying a large number of redox tracers.
For example, ultrasensitive electrical DNA detection was reported on the basis of polystyrene beads impregnated with the ferrocenecarboxaldehyde redox marker [36]. An amplified electrochemical sandwich immunoassay based on a polyelectrolyte-coated ferrocene microcrystal was also reported [37]. Capturing of the antibody-conjugated ferrocene microcystal was followed by release of a large amount of the redox marker through the capsule wall (by a releasing agent), and led to a highly sensitive amperometric biodetection. The successful clinical realization of these ultrasensitive bioelectronic detection schemes requires proper attention to non-specific adsorption issues that commonly control the detectability of bioaffinity assays. Nanoparticle-induced changes in the conductivity across a microelectrode gap were exploited for highly sensitive electronic detection of DNA hybridization [38].
The capture of the nanoparticle-tagged DNA targets by probes immobilized in the gap between the two closely spaced microelectrodes, and a subsequent silver precipitation, resulted in a conductive metal layer across the gap, and led to a measurable conductivity signal. Such hybridization-induced conductivity signals, offered low detection limits down to the 0.5pM level. Inorganic nanocrystals offer an electrodiverse population of electrical tags as needed for multiplexed clinical testing. The use of different inorganic-nanocrystal tracers for a multitarget electronic detection of proteins [39] or DNA[40].
Four encoding nanoparticles (cadmium sulfide, zinc sulfide, copper sulfide, and lead sulfide) were thus used to differentiate the signals of four proteins or DNA targets in connection with sandwich immunoassay and hybridization assay, respectively, along with stripping voltammetry of the corresponding metals. Each binding event thus yielded a distinct voltammetric peak, whose size and position reflected the level and identity, respectively, of the corresponding antigen or DNA target. The concept can be readily scaled up and multiplexed by using a parallel highthroughput automated microwell operation, with each microcavity capable of measuremsnts.
Fig c. Simultaneous monitoring of multiple proteins in connection to different inorganic nanocrystal tags and electrochemical stripping transduction [43]
Conclusion:
One-dimensional nanostructures are extremely attractive for a wide range of bioelectronic sensing applications. The ability to modify nanowires and nanotubes with biological recognition elements imparts high selectivity onto devices based on 1-D nanomaterials.
While several novel sensing concepts based on 1-D nanowires have been presented, incorporating these materials into routine functional devices remains a challenge. The successful bioelectronics utility of 1-D nanostructures requires new nanofabrication capabilities and proper attention to the interconnection challenge, involving reproducible positioning of nanowires and nanotubes between closely-spaced microelectrodes. Such attention to the nanotechnology/ microtechnology interface is essential for assembling nanosensors into functional integrated devices. Proper attention should be given also to the interface of these devices with the real world (i.e., to sample delivery issues).
Ultimately, such activity will lead to powerful sensor arrays for parallel real-time monitoring of multiple analytes. The creation of such biosensor arrays requires new methods for confining different biomolecules onto closely spaced 1-D nanostructures. A wide range of newly introduced nanomaterials is expected to expand the realm of nanomaterial-based biosensors. Such nanomaterials-based electrochemical devices are expected to have a major impact upon clinical diagnostics, environmental monitoring, security surveillance, or for ensuring our food safety. It is only a matter of time before such protocols are used for routine diagnostic applications.
References:
[1] Introduction to Nanotechnology, Wiley, 2003. [2] Analyst, 2004, 129, 672. [3] Acc. Chem. Res., 1995, 28, 61. [4] ChemPhysChem, 2001, 2, 79. [5] Electroanalysis, 1997, 9, 661. [6] J. Am. Chem. Soc., 2003, 125, 9006. [7] Electrochem. Commun., 2003, 5, 408 [8] Angew Chem. Int. Ed., 2004, 43, 2113. [9] Science, 2003, 299, 1877. [10] J. Am. Chem. Soc., 2003, 125, 2408.
[11] Electrochem. Commun., 2002, 4, 743 [12] Anal. Chem., 2004, 76, 1083 [13] Electroanalysis, 2004, 16, 54. [14] NanoLett, 2004, 4, 1785. [15] NanoLett, 2004, 4, 1237. [16] Trends Anal.Chem. 2005, 21, 213–219. [17] Biosens. Bioelectron. 2005, 20, 1461–1470. [18] Anal. Biochem., 1979, 94, 22–28. [19] Clin. Chem., 1995 41, 1378–1384. [20] Anal. Chem.,2003, 75, 125. [21] Anal. Chim. Acta, 2003, 497, 75–81. [22] Analytical Electrochemistry.2001, Wiley, New York, [23] Curr. Pharm. Biotechnol. , 2010, 5, 45–67. [24] Anal. Chem., 2009, 75, 1116–1122. [25] Chem. Commun., 2011, 4267. [26] Science 2010, 300, 11–15. [27] Nano Lett., 2010 4, 51–55. [28] Proc. Natl. Acad. Sci. U.S.A. , 2007, 101, 14017–14022. [29] Nat. Biotechnol., 2005 23, 1294. [30] J. Am. Chem. Soc., 2007 127, 496–497. [31] Chem. Phys. Chem., 2006 5, 1085–1091. [32] Stripping Analysis., 1985 VCH, New York [33] Anal. Chem. , 2001, 72, 5521.
[34] Nucl. Acids Res. , 2007, 28,3011–3016. [35] Electroanalysis, 2004 16,
101–105. [36] Langmuir, 2009 19, 989–992 [37] Anal. Chem., 2005 77, 2835–2841. [38] Science, 2002, 295, 1503–1506. [39] Anal. Chem.,2011, 76, 7126–7130 [40] J. Am. Chem. Soc., 2003 125, 3214–3215. [41] Analyst, 2005, 130, 421–426 [42] Biosens. Bioelectron. 2006, 21, 1887-1892. [43] Anal. Chem. , 2003, 81, 7121–7129.